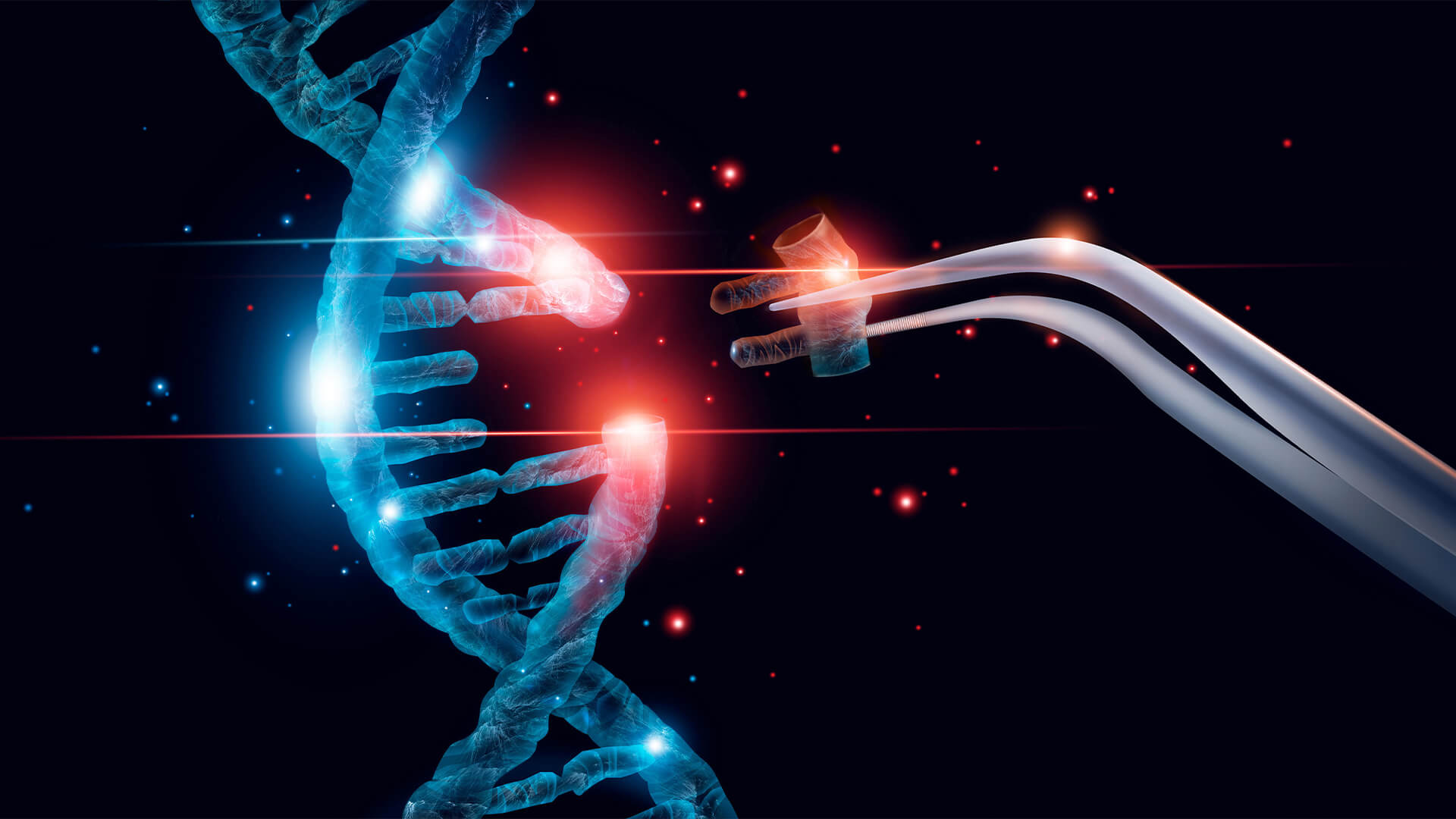
Clustered regularly interspaced short palindromic repeat — or CRISPR — gene editing technologies enable a huge level of dexterity for scientists when it comes to precise genome manipulation and diagnostics, meaning that the ways they can alter DNA and turn genes on or off are greater than ever before. A range of developments in the CRISPR gene editing space have paved the way for this progression.
Here, we’ll explore why CRISPR/Cas9 is ideal for gene inactivation and why base editing and prime editing are better suited to treating genetic disorders than disruptive approaches. We’ll also touch on the importance of nucleic-acid-based diagnostics and CRISPR/Cas systems, including Cas13.
Those who are interested in CRISPR diagnostics can find further information on these topics in the life sciences journal BioTechniques.
CRISPR/Cas9
Out of the many CRISPR technologies available to scientists, one that is often used is the CRISPR/Cas9 technology. While targeting the Cas9 enzyme to a genomic sequence is a relatively precise process, the cell’s repair of the resulting double-stranded cut isn’t precise. Therefore, the CRISPR/Cas9 technology is better suited to gene inactivation than repair. This is because a method known as non-homologous end-joining mediates CRISPR/Cas9 repairs, which can be muddied by minute insertions or deletions.
Gene Correction: Base Editing and Prime Editing
Gene correction is usually a more effective approach than disruption when it comes to treating genetic diseases. David Liu, one of Harvard University’s chemical biologists, has collaborated with his team to conceptualize two gene correction approaches: prime editing and base editing. These editing approaches leverage CRISPR’s precise targeting, limit Cas9’s ability to cut DNA at a selected site, and cut a single DNA strand, which is safer and less disruptive for cells than alternative approaches.
Base editing merges a catalytically impaired form of Cas9 with an enzyme that stimulates the chemical conversion of one nucleotide to another. For example, an enzyme might convert cytosine to thymine or adenine to guanine. Since 2016, when base editing was first described, this type of editing has quickly progressed toward clinical use. Liu’s company Beam Therapeutics has received approval from the U.S. Food and Drug Administration to pilot the editing approach on sickle-cell disease patients. That said, this kind of editing only makes some base-to-base changes accessible.
Meanwhile, prime editing is a newer development on the CRISPR gene editing scene. This editing approach links Cas9 to a type of enzyme termed reverse transcriptase and includes the desired edit in a genomic sequence using a modified guide RNA. A multistage biochemical process sees these components copy the guide RNA into DNA that replaces the targeted genome sequence.
Although prime editing hasn’t developed as much as base editing, new iterations of this process are emerging. For example, Hyongbum Henry Kim, one of Yonsei University College of Medicine’s genome editing specialists, has worked with his team to prove they can achieve up to 16% efficacy in implementing this kind of gene editing to correct retinal gene mutations in mice.
On top of this, Liu and his team have discovered that they can use prime machinery to assist the insertion of gene-sized DNA sequences into the genome. With this machinery, they can offer a safer, better controlled gene therapy. While the process isn’t fully efficient, even a small repair can go a long way.
The Importance of Nucleic-Acid-Based Diagnostics
Quick, accurate diagnoses and treatment recommendations are essential for optimal patient outcomes. And nucleic-acid-based biomarkers that are associated with a disease can be crucial to diagnostics. This is because scientists can amplify RNA and DNA from trace amounts, which facilitates their highly specific detection through the pairing of complementary nucleotides.
As a result, nucleic-acid-based diagnostics have raised the bar for diagnosing several chronic and acute conditions, especially conditions that infectious diseases cause. Precise, fast nucleic-acid-based testing is especially important during outbreaks of infectious diseases, such as Covid-19, to control the spread of the disease effectively.
Nucleic-acid-based diagnostics that rely on quantitative polymerase chain reaction (qPCR) or sequencing are now particularly common, especially in clinical labs. The versatility, robustness, and sensitivity of PCR have made this technology ideal for detecting DNA and RNA biomarkers. Diagnostics based on nucleic acid detection are often the most sensitive and specific. However, most assays require costly equipment and trained personnel.
With isothermal nucleic acid amplification, there is no need for thermal cyclers. However, non-specific amplification can cause lower detection specificity. Scientists can perform additional readouts, utilizing fluorescent probes, molecular beacons, or oligo strand-displacement probes to improve the specificity.
But scientists often need technologies that combine the cost-effectiveness and ease of use of isothermal amplification with PCR’s diagnostic accuracy. Ideally, diagnostics should also have single-nucleotide specificity, which is pivotal to the identification of mutations conferring resistance against antiviral drugs and antibiotics. CRISPR-based diagnostics have the potential to meet these criteria.
The Importance of CRISPR/Cas Systems
There are several CRISPR/Cas systems among bacteria and archaea that scientists can utilize. These systems are all dependent on CRISPR RNA (crRNA), which enables Cas proteins to recognize and cleave nucleic acid targets. Scientists can program crRNA toward an RNA or DNA region of interest through hybridization to a complementary sequence. In some systems, this sequence may be restricted to the proximity of a protospacer flanking sequence or protospacer adjacent motif (PAM).
So far, scientists have utilized CRISPR/Cas in a variety of applications, including the
- Detection of nucleic acids.
- Bioimaging of nucleic acids.
- Targeted editing of genomes, transcriptomes, and epigenomes.
- Recording of cellular events.
CRISPR-based diagnostics are progressing at speed by building on CRISPR technologies’ specificity, ease of use, and programmability. As a result, scientists are working ever closer to creating nucleic-acid-based point-of-care (POC) diagnostic tests, which could become standard in clinical care.
Since the beginning of CRISPR gene editing, the number of CRISPR/Cas systems available has quickly grown. Today, there are two classes, six types, and several more subtypes of these systems. Scientists categorize these classes, types, and subtypes by the nature of the ribonucleoprotein effector complex.
While multiple effector proteins characterize class 1 systems, class 2 systems incorporate a crRNA-binding protein. As these systems are easier to reconstruct, they’re used more often in diagnostics. Class 2 systems include enzymes with collateral activity, which form the mainstay of a variety of CRISPR-based diagnostic assays. However, scientists have engineered some class 1 systems with diagnostics, either with the native type three complex or components of the class 2 system.
CRISPR/Cas13
The CRISPR/Cas system’s role as a bacterial immune system against viral infections gives it capacity for the precise cleavage of specific nucleic acid sequences. Many early adopters of CRISPR gene editing considered the system’s applicability to viral diagnostics because of this capacity. That said, each Cas enzyme is different and has different applications. For example, while Cas9 is the best enzyme for CRISPR-based genome manipulation, several CRISPR-based diagnostic approaches leverage Cas13, a family of RNA-targeting molecules that the molecular biologist Feng Zhang identified with his team in 2016.
Cas13 utilizes its RNA guide to identify an RNA target by base-pairing, and then activate a ribonuclease activity. Scientists can harness this ribonuclease activity as a diagnostic tool with a reporter RNA. Aside from cutting the RNA targeted by the guide RNA, Cas13 also performs “collateral cleavage” on any nearby RNA molecules.
Several Cas13-based diagnostics utilize a reporter RNA that tethers a fluorescent tag to a quencher molecule to impede fluorescence. Cas13 activates when it recognizes viral RNA. Cas13 then cuts the reporter and releases the fluorescent tag from the quencher, generating a detectable signal.
Some viruses leave a signature that is so strong scientists can achieve detection without amplification, simplifying point-of-care diagnostics. As an example, at the Gladstone Institute of Virology, San Francisco, Jennifer Doudna and Melanie Ott provided a demonstration of a rapid, amplification-free, nasal-swab-based CRISPR/Cas13 test for SARS-CoV-2 using a smartphone camera.
RNA-amplification processes can improve sensitivity for trace viral sequences. For example, Pardis Sabet, a Broad Institute of Massachusetts Institute of Technology and Harvard geneticist, worked with her team to develop a microfluidic system that screens for pathogens while using amplified genetic material from a few microliters of sample. The research team developed CRISPR-based detection tools that can identify more than 169 human viruses at the same time.
Scientists can also make the most of other Cas enzymes, such as Cas12, which exhibits similar properties to Cas13 but targets DNA instead of RNA. By utilizing a range of Cas proteins, scientists can identify a wider variety of pathogens and effectively diagnose diseases.